Research finds small brains can accomplish big things
Smaller networks and smaller brains can perform more complicated computations than scientists previously thought.
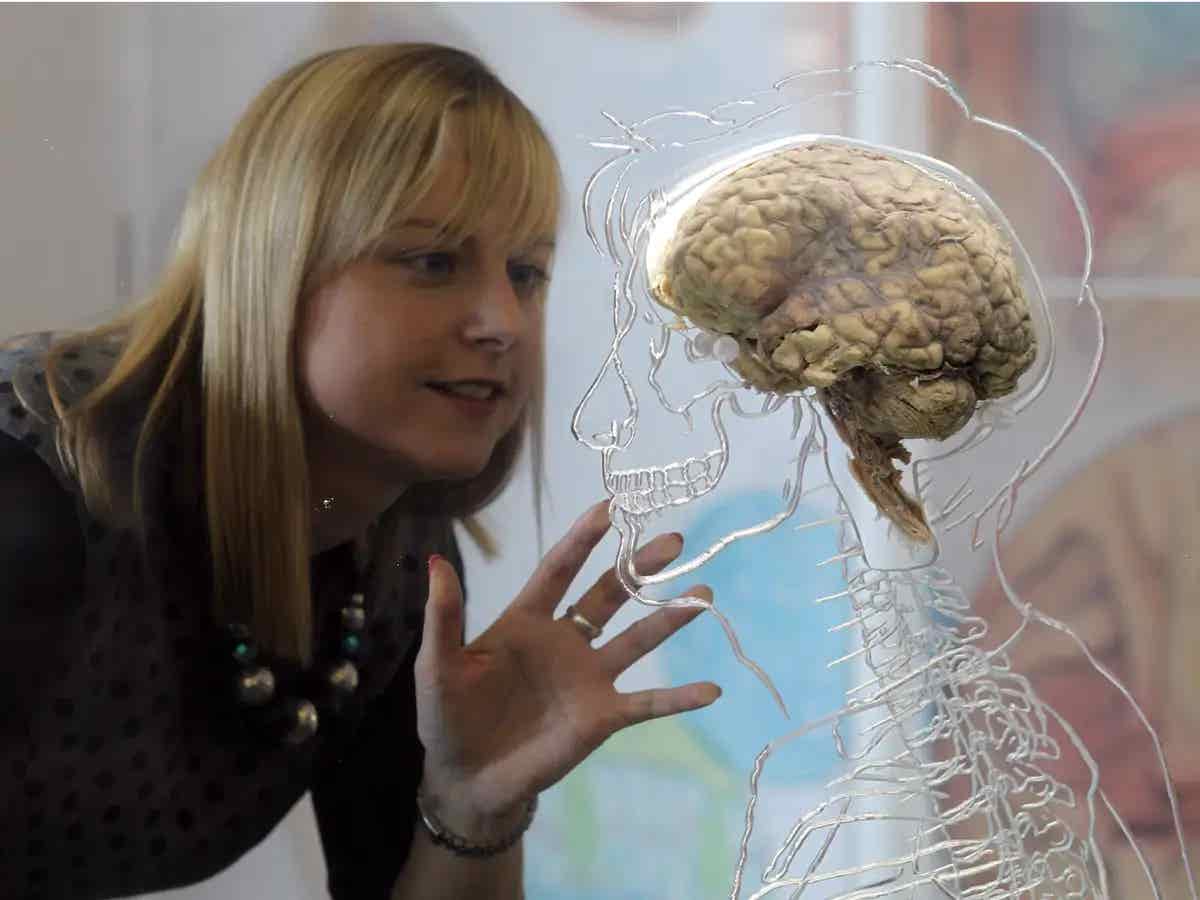
For decades, neuroscientists theorized that networks of neurons called ring attractor networks were essential for an internal compass. (CREDIT: CC BY-SA 4.0)
The brain's ability to track orientation and position is essential for memory, navigation, and decision-making. Traditionally, scientists believed that accurate tracking of such variables required large, complex neural networks. However, new research into fruit flies’ brains has upended this assumption, revealing how small neural networks can accomplish tasks once thought impossible for their size.
For decades, neuroscientists theorized that networks of neurons called ring attractor networks were essential for an internal compass. These networks create a "bump" of activity, which represents orientation and can persist even without external cues. Such systems were thought to require many neurons to maintain precision and accuracy. Smaller networks, by contrast, were assumed to be prone to errors like drifting or misalignment.
However, this theory was challenged when researchers discovered that fruit flies possess an internal compass built on a much smaller network. “The fly’s compass is very accurate, but it’s built from a really small network, contrary to what previous theories assumed,” says Ann Hermundstad, a group leader at HHMI’s Janelia Research Campus.
Marcella Noorman, a postdoctoral researcher at Janelia, sought to uncover how this small network achieved such precision. Her findings, published in the journal, Nature Neuroscience, not only solve this mystery but also reshape our understanding of brain computation.
Ring attractor networks operate by creating a circular map of activity. Each neuron in the network corresponds to a specific orientation. Neurons tuned to similar orientations excite one another, while those with dissimilar orientations inhibit each other. This connectivity enables the system to maintain a stable representation of direction and to update it when the animal moves.
In large networks, these dynamics are precise and resilient, maintaining the activity “bump” without input and updating it smoothly based on self-motion. However, in small networks, these properties were assumed to degrade. Discrete basins of activity could cause errors, such as the activity bump drifting to stereotypical orientations or failing to integrate small changes in motion.
Despite this, fruit flies defy expectations. Their compass neurons, located in a brain region called the central complex, exhibit dynamics characteristic of ring attractor networks. Observations show that these neurons maintain stable activity bumps that track the fly’s movements even in darkness.
“Smaller networks and smaller brains can perform more complicated computations than we previously thought,” Noorman explains. “But to do so, the neurons must be connected much more precisely than in larger systems.”
Related Stories
To understand how small networks achieve this, Noorman stripped down existing models of ring attractor networks, removing additional complexities like biophysical properties. Initially, she believed a small network could not generate a stable ring attractor. But after failing to prove this, she reconsidered her hypothesis.
By flipping her perspective, Noorman demonstrated that a small network with as few as four neurons could function as a ring attractor. The key was in fine-tuning the connections between neurons. Experimental data from fruit flies confirmed this theory.
“There’s a trade-off between how many neurons you use for computation and how carefully you have to connect them,” Noorman says. Smaller networks rely on precision, whereas larger networks can afford less exact connectivity due to redundancy.
Using two-photon calcium imaging, researchers examined the activity of compass neurons in flies tethered to an air-supported ball. This setup allowed scientists to study how these neurons tracked orientation in darkness. The results were surprising.
The flies’ internal compass maintained orientation without significant drift, even in the absence of external sensory input. The activity bump moved continuously and accurately aligned with the fly’s movements, showing no evidence of errors expected from discrete systems.
“Despite its small size, the head direction system’s performance is remarkable,” Hermundstad notes.
These findings expand the known capabilities of small neural networks. Even with limited resources, small networks can represent continuous variables with high precision. This raises intriguing questions about how larger brains might use similar principles to perform even more complex tasks.
Fruit flies have provided neuroscientists with a model that demonstrates how small systems can overcome apparent limitations. The precise connectivity observed in these networks may explain their ability to achieve continuous representation without error. Researchers now aim to explore whether similar principles are at work in other species with compact neural architectures.
The fruit fly’s compact yet powerful compass highlights an important trade-off in neural computation: precision in connectivity versus the number of neurons. This discovery could have broad implications for neuroscience, robotics, and artificial intelligence. By understanding how small networks achieve such efficiency, scientists could design better algorithms for navigation or memory in machines.
Next steps include investigating how additional features, like sensory inputs or biophysical details, enhance the robustness of these networks. Researchers also plan to explore whether this architecture serves as a foundation for more complex computations in larger systems.
“This work expands the computational repertoire of small networks and raises the possibility that larger networks could represent more and higher-dimensional variables than previously thought,” Noorman says.
By expanding on these principles, researchers hope to uncover new insights into how brains of all sizes process information. Larger networks may use small-scale attractor principles to manage complex and multidimensional tasks, further connecting these discoveries to broader neural functions.
As scientists delve deeper into the fly’s head direction system, they aim to determine how sensory cues interact with these networks. For example, how do external stimuli adjust or refine the internal representation of direction? Such research could lead to advances in understanding the balance between internal neural computation and external environmental inputs.
Noorman’s journey from mathematics to neuroscience illustrates the power of interdisciplinary approaches in science. Translating biological questions into mathematical models allowed her to challenge and ultimately overturn assumptions about small networks.
“The fly’s head direction system was the first example of neural activity I’d ever seen. Figuring out how it works has been a fun challenge,” she reflects.
The ability of small brains to perform tasks thought to require larger systems reveals a fundamental adaptability in neural computation. These findings underscore the efficiency of evolution, showing that even tiny networks can achieve extraordinary feats. By studying these systems, researchers are not only advancing neuroscience but also paving the way for innovations in technology and artificial intelligence.
Ultimately, the fruit fly’s internal compass demonstrates that size is no limitation to complexity. The lessons learned from these small networks may illuminate the fundamental principles of computation that underlie all brain functions, from simple organisms to humans.
This research invites us to reconsider what is possible in both nature and technology, proving that even the smallest brains can hold big ideas.
Note: Materials provided above by The Brighter Side of News. Content may be edited for style and length.
Like these kind of feel good stories? Get The Brighter Side of News' newsletter.
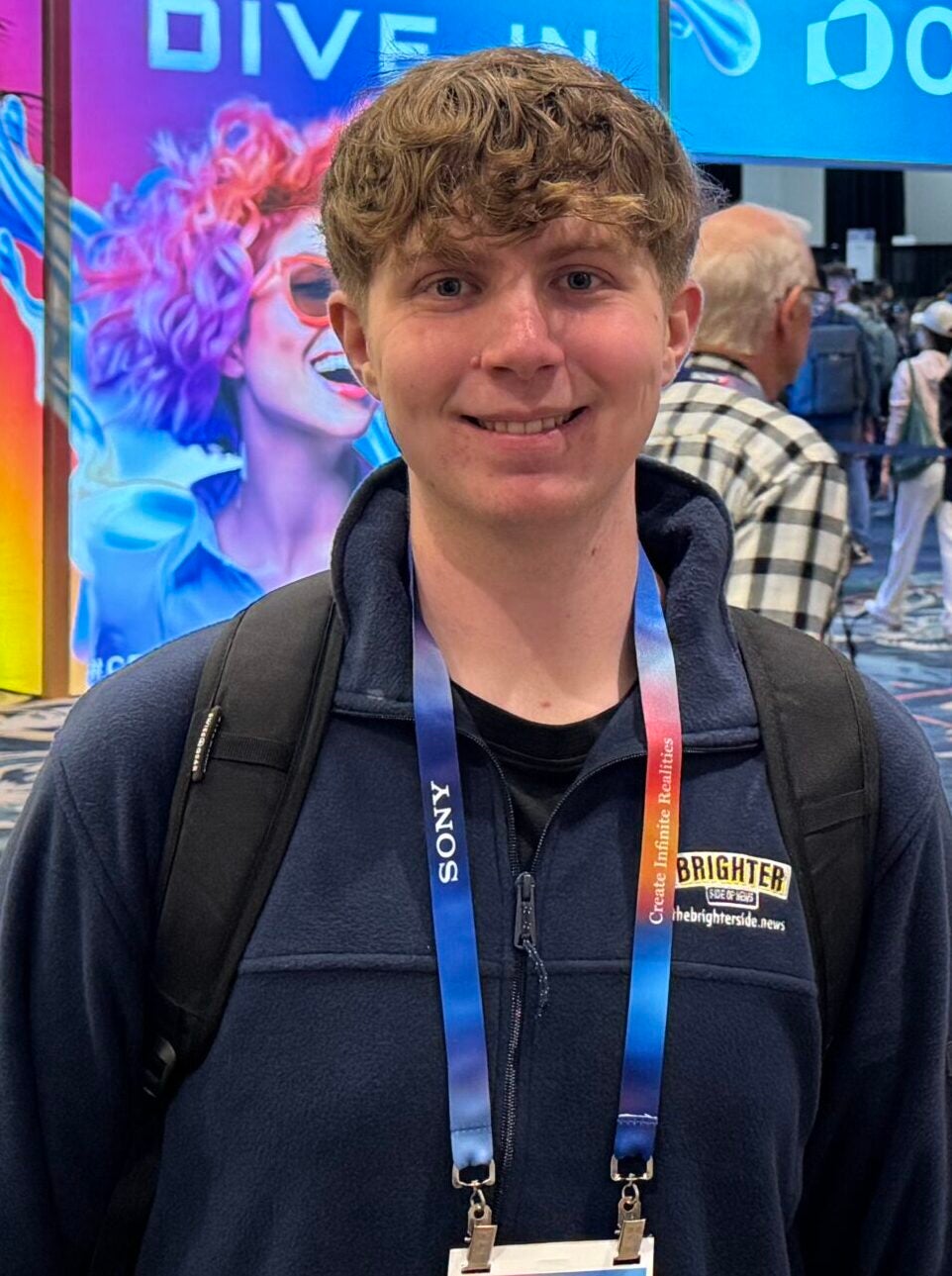
Joshua Shavit
Science & Technology Writer | AI and Robotics Reporter
Joshua Shavit is a Los Angeles-based science and technology writer with a passion for exploring the breakthroughs shaping the future. As a contributor to The Brighter Side of News, he focuses on positive and transformative advancements in AI, technology, physics, engineering, robotics and space science. Joshua is currently working towards a Bachelor of Science in Business Administration at the University of California, Berkeley. He combines his academic background with a talent for storytelling, making complex scientific discoveries engaging and accessible. His work highlights the innovators behind the ideas, bringing readers closer to the people driving progress.