Physicists capture historic first look at the internal structure of a neutron
Physicists used a new detector to measure deeply virtual Compton scattering on the neutron for the first time, revealing inner nucleon structure.

First DVCS measurement on the neutron reveals quark contributions to spin, unlocking secrets of nucleon structure. (CREDIT: Shutterstock)
In the world of nuclear physics, protons often take the spotlight. But now, for the first time, the neutron has stepped into it. A recent experiment using the U.S. Department of Energy’s Jefferson Lab has revealed the inner structure of the neutron in ways never seen before.
This milestone gives scientists a new tool to unlock one of physics’ biggest puzzles: how the spin of particles like protons and neutrons—called nucleons—really works.
Researchers have known for years that nucleons are built from smaller particles, called quarks and gluons. These are collectively known as partons. But how these partons are arranged inside the nucleon, and how they add up to produce the nucleon’s spin, remains unclear. Past experiments showed that quark spins only account for about 20% to 30% of the total spin, sparking what’s known as the “nucleon spin crisis.”
Now, scientists are using a tool called deeply virtual Compton scattering, or DVCS, to look deeper. This method offers a way to peek inside a nucleon by shooting a high-energy electron at it and watching how the nucleon absorbs energy and emits a photon without breaking apart. Tracking what comes out of this interaction tells researchers a lot about what’s going on inside.
A New View Into the Neutron
The breakthrough came from a team of scientists at the U.S. Department of Energy’s Thomas Jefferson National Accelerator Facility who figured out how to study DVCS not just on protons—but on neutrons too. That wasn’t easy. Neutrons, unlike protons, are harder to detect after a DVCS event. They often fly out at steep angles where detectors couldn’t reach—until now.
In 2007, Silvia Niccolai, a physicist with the French National Centre for Scientific Research (CNRS), began developing a new way to catch those elusive neutrons. She designed a special tool, the Central Neutron Detector, to be used with Jefferson Lab’s powerful CLAS12 particle detector.
Building that detector took years. Niccolai’s team at IJCLab, a joint lab in Orsay, France, worked with students and postdocs to complete the device in 2015. It was installed at Jefferson Lab two years later, in 2017. Pierre Chatagnon, then a Ph.D. student at Paris-Saclay University, helped install and calibrate it. He’s now a postdoctoral researcher at Jefferson Lab.
Related Stories
Between 2019 and 2020, the Central Neutron Detector ran alongside the CLAS12 system and finally began collecting DVCS data on neutrons. The researchers thought they were ready to dig into their results—until they discovered an issue.
“We detected the neutron for the first time in this type of reaction, and it’s a quite important result for the study of nucleons,” said Niccolai.
Fixing the Signal
The detector had been built to ignore charged particles, such as protons. But during analysis, the team realized that some protons were sneaking through the system undetected. These proton “contaminants” risked spoiling the neutron data.
That’s when physicist Adam Hobart, a researcher at IJCLab, stepped in. He used machine learning to tell the difference between real neutron signals and fakes. His tool combed through the data and cleaned it up.
“This was solved thanks to Adam's experience using machine learning techniques,” Niccolai said. “He developed a ML-based tool to discriminate fake signals from real neutrons, and this was vital to achieve our final results.”
Thanks to this fix, the team could say with confidence that they were measuring real DVCS events involving neutrons. This was critical. When the neutron isn’t directly detected, it’s harder to be sure what kind of reaction occurred. Detecting the actual neutron made their data far more reliable.
“If you’re not detecting the neutron, you have a certain range of possibilities of the process that is happening,” Hobart said. “Then you have less precision in the observables that you would measure later.”
Mapping What’s Inside
The reason DVCS is so powerful is because it connects what happens in a lab to what’s happening inside the nucleon. This connection is made using a theoretical tool called generalized parton distributions, or GPDs. GPDs allow physicists to map how partons are arranged inside a nucleon, including both their positions and how fast they move.
There are four main GPDs, each providing different information. One of them, called GPD E, is among the least understood. It’s also one of the most important. GPD E helps explain how the motion of quarks contributes to the overall spin of a nucleon.
By using a polarized electron beam—where all the electrons’ spins point in the same direction—the researchers could measure an effect called beam-spin asymmetry. This helped them isolate GPD E with high precision.
“The GPD E is very important because it can give us information into the spin structure of nucleons,” Niccolai said.
GPDs depend on several variables: the momentum of the quarks, the angle of the scattered particles, and the amount of energy exchanged. These variables are packed into complex mathematical formulas. But the end result is simple: when you know your GPDs, you know how the nucleon is built on the inside.
And that’s the goal—building a full picture of how spin arises from the sum of its parts.
The First Flavor Separation
The neutron data also gave the scientists a bonus. It let them look at up and down quarks separately. These are the two “flavors” of quarks found in protons and neutrons. Protons have two up quarks and one down quark; neutrons have two down quarks and one up.
By combining the new neutron DVCS data with older proton DVCS data, researchers could, for the first time, separate the imaginary parts of two key GPDs—E and H—by quark flavor. This means they can now see how each flavor of quark contributes differently to nucleon spin.
This was a joint effort. Theorists Maria Čuić and Krešimir Kumerički provided critical theoretical support to make the flavor separation work. And the full CLAS collaboration was essential in collecting and analyzing the mountain of data needed for this result.
“We must give gratitude to the whole CLAS Collaboration,” Hobart said. “Taking and processing the data is collaborative work.”
The researchers now plan to collect more neutron DVCS data to refine their findings. But even now, this experiment stands as a landmark in nuclear physics.
“This first result is major,” Niccolai said. “It feels like the completion of a cycle and a lifetime achievement because this is the first project that I took full responsibility for in my career. Finally arriving at a physically meaningful result and having it published feels like I’ve had another baby.”
This first glimpse into the neutron’s structure marks the beginning of a new chapter in the decades-long quest to solve the spin crisis. With better tools and clearer signals, the pieces are finally starting to fall into place.
Research findings are available online in the journal Physical Review Letters.
Note: The article above provided above by The Brighter Side of News.
Like these kind of feel good stories? Get The Brighter Side of News' newsletter.
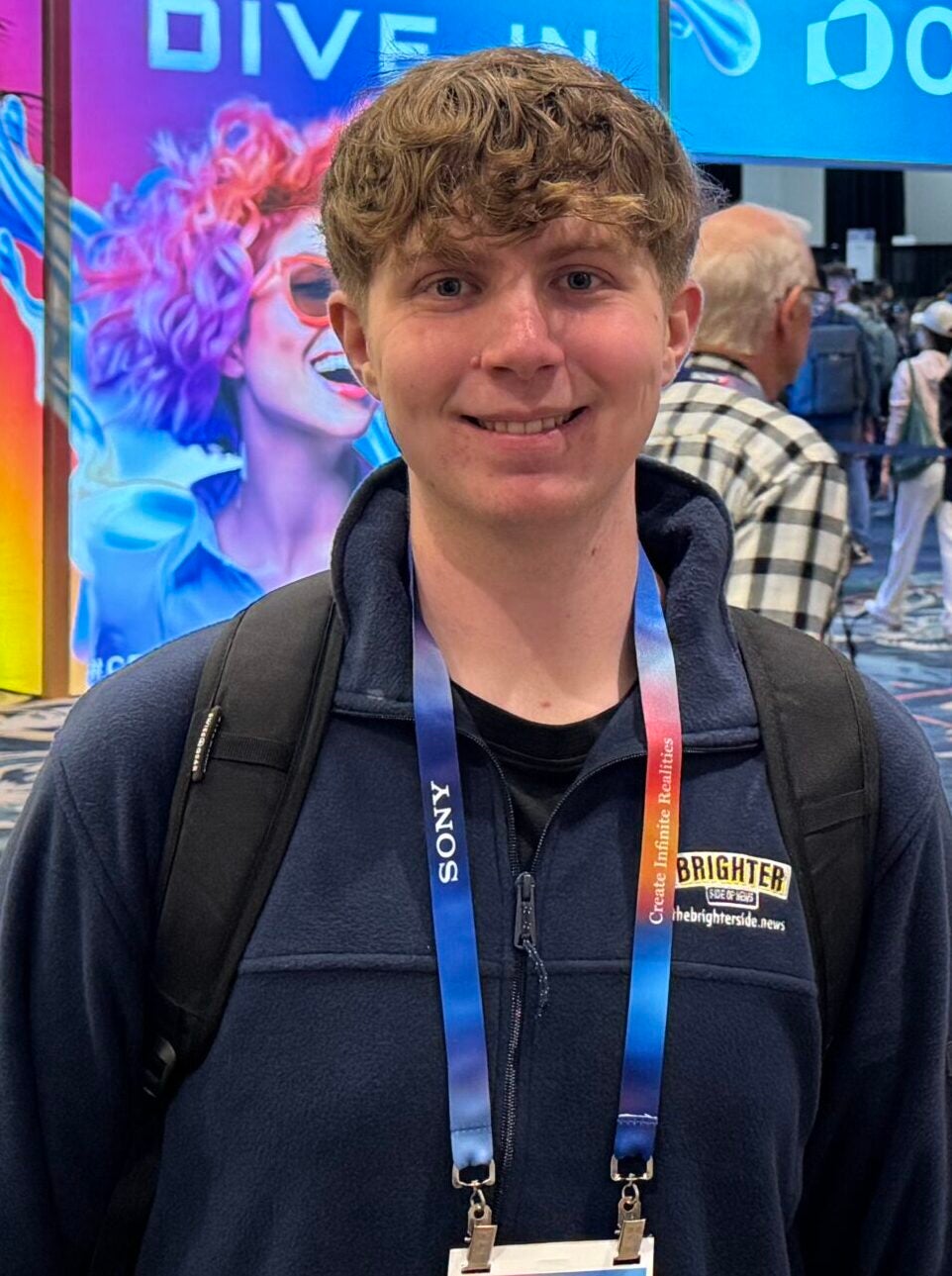
Joshua Shavit
Science & Technology Writer | AI and Robotics Reporter
Joshua Shavit is a Los Angeles-based science and technology writer with a passion for exploring the breakthroughs shaping the future. As a contributor to The Brighter Side of News, he focuses on positive and transformative advancements in AI, technology, physics, engineering, robotics and space science. Joshua is currently working towards a Bachelor of Science in Business Administration at the University of California, Berkeley. He combines his academic background with a talent for storytelling, making complex scientific discoveries engaging and accessible. His work highlights the innovators behind the ideas, bringing readers closer to the people driving progress.