Groundbreaking high-speed 3D bioprinter revolutionizes drug discovery
Discover the groundbreaking 3D bioprinter using sound and light to replicate human tissues quickly and accurately for advanced medical research.
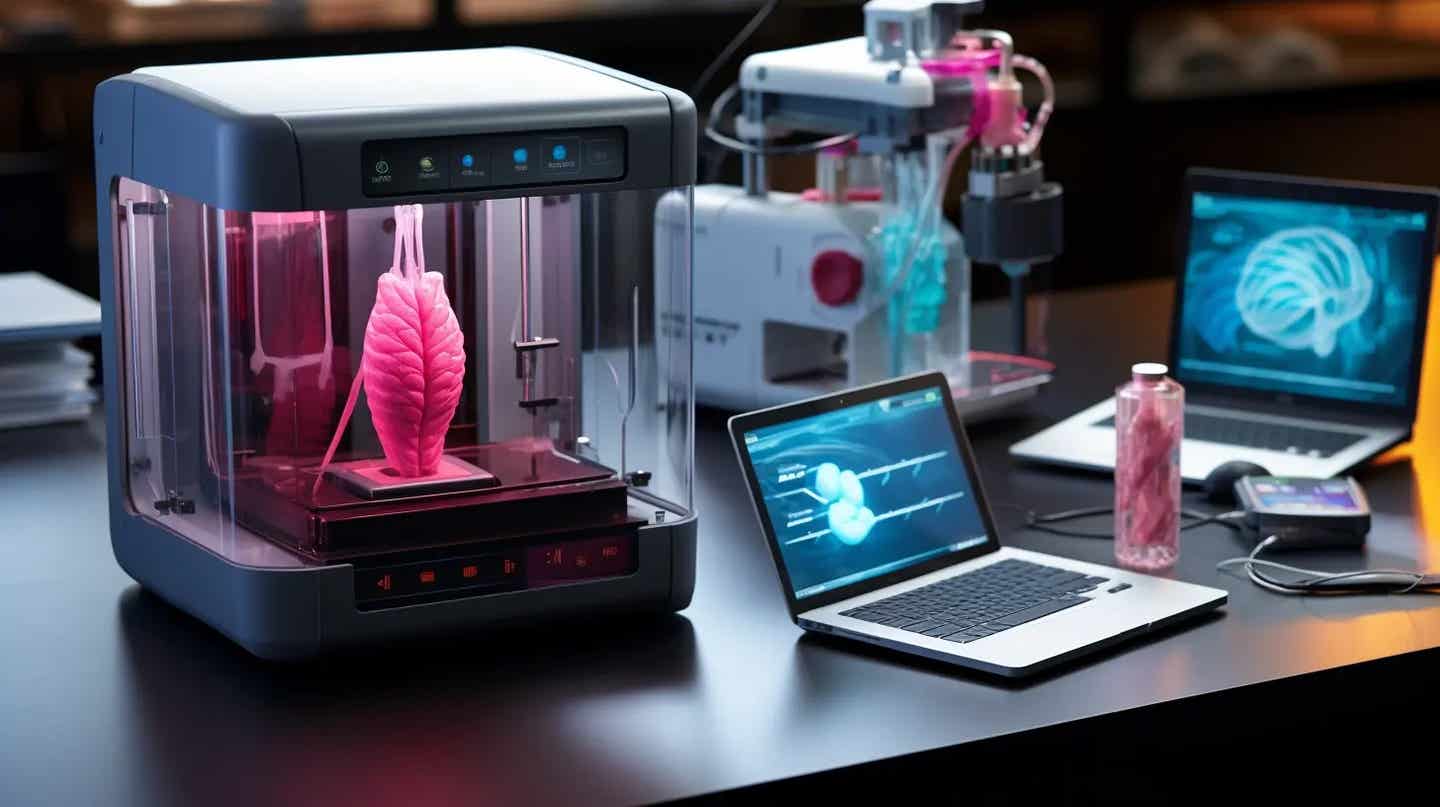
Example of 3D-bioprinter offering faster, more accurate tissue replication. (CREDIT: CC BY-SA 4.0)
University of Melbourne researchers have developed a revolutionary 3D bioprinting technology that could transform medical research and the development of new treatments. Unlike traditional bioprinters, which rely on layer-by-layer printing, this innovative bioprinter leverages sound waves and light to create complex cellular structures in seconds.
With this approach, biomedical engineers can more accurately replicate human tissues, from soft brain matter to denser structures like cartilage and bone. This breakthrough opens doors for new applications in cancer research, pharmaceutical development, and regenerative medicine.
Traditional bioprinters use a layer-based approach where each layer is printed one at a time. While effective for smaller structures, this method is time-consuming and places living cells at risk, as long printing times can decrease cell viability. Moreover, transferring these fragile cell structures for further study requires delicate handling, which can compromise their integrity.
To address these limitations, the research team designed a system known as Dynamic Interface Printing (DIP). DIP utilizes a hollow print head with an air-liquid meniscus at its base, which serves as the printing interface. As visible light passes through a glass window atop the print head, structures are solidified at the air-liquid boundary.
The DIP print head submerges in a prepolymer liquid, trapping air and forming a stable meniscus, or boundary layer, where structures are formed. Using this system, researchers can create complex 3D shapes without requiring layer-by-layer assembly or highly specialized optical systems.
Associate Professor David Collins, head of the Collins BioMicrosystems Laboratory at the University of Melbourne, emphasized the system's advantages in cell positioning. “In addition to drastically improving print speed, our approach enables a degree of cell positioning within printed tissues,” he explained.
According to Collins, the precise positioning of cells is a crucial factor that distinguishes DIP from other bioprinting methods. “Incorrect cell positioning is a big reason most 3D bioprinters fail to produce structures that accurately represent human tissue,” he added.
Related Stories
Collins compared DIP's benefits to the precise engineering required for a car: “Just as a car requires its mechanical components to be arranged precisely for proper function, so too must the cells in our tissues be organized correctly.” Unlike other bioprinters, which depend on cells to align naturally, DIP uses acoustic waves generated by vibrating bubbles to actively guide cells into their intended positions within the printed structure.
This acoustic modulation is a key feature of DIP, distinguishing it from slower, traditional methods. By vibrating the air-liquid interface, DIP creates capillary-gravity waves that enhance material flow. This process increases print speed, fidelity, and the structural integrity of printed tissues.
Particle Image Velocimetry (PIV) tests demonstrated fluid flow rates of over 10 mm per second at the interface, with speeds up to 45 mm per second achievable under certain conditions. These high rates support faster printing, enabling centimetre-scale constructs in under a minute.
Callum Vidler, a PhD student and lead author on the DIP project, highlighted the impact of this rapid fabrication capability. “Biologists recognize the immense potential of bioprinting, but until now, it has been limited to applications with a very low output,” Vidler said.
The technology, which can print 350 times faster than conventional methods, offers major advancements in speed, precision, and consistency. Vidler added that the response from the medical research community has been enthusiastic.
“So far, we've engaged with around 60 researchers from institutions including the Peter MacCallum Cancer Centre, Harvard Medical School, and the Sloan Kettering Cancer Centre, and the feedback has been overwhelmingly positive.”
One of the biggest technical hurdles in bioprinting is creating structures that are both precise and viable on a cellular level. With DIP’s optical system, the print interface is dynamically adjusted using acoustic waves, allowing researchers to position cells accurately without requiring complex adjustments in the material or setup.
In essence, this system replaces layer-based printing with a boundary-driven process where light and sound work together to create tissue structures with remarkable cellular detail and integrity.
Another standout aspect of DIP is its capacity to handle a wide variety of materials. Previous optical-based methods for rapid printing have been limited by requirements for material transparency, which restricts the use of many cell-based or particulate bioinks.
DIP’s design minimizes these constraints, as it operates with an air-liquid boundary instead of a fully immersed material. This flexibility allows researchers to use common biologically relevant materials like poly(ethylene glycol) diacrylate (PEGDA) and gelatin methacryloyl (GelMA) with high printing speeds and resolution.
The DIP system also offers significant advantages for creating large or opaque structures, something current volumetric printing systems struggle with due to light scattering and absorption issues. In a demonstration of DIP’s capabilities, the team printed a tricuspid valve using a custom bioink containing norbornene-functionalized sodium alginate. Despite the ink’s opacity, DIP successfully created the valve in just 33 seconds with detailed internal features.
Moreover, DIP’s acoustic modulation enables further control over the distribution of granular additives like cells or particles, minimizing sedimentation. This capability is essential for creating bioengineered tissues that require uniform cell distribution. DIP’s mixing capability can enhance encapsulation efficiency, allowing for denser, more complex tissue scaffolds with fewer additives.
Beyond printing speed and precision, the DIP system is adaptable to different container shapes, meaning it is not constrained by the size or geometry of the print vessel. This adaptability allows for greater design flexibility, enabling the creation of intricate, multi-material structures in shapes previously unachievable with conventional methods.
The benefits of DIP extend beyond cancer research, with potential applications in drug testing, tissue engineering, and regenerative medicine. Researchers can use DIP to replicate human organs and tissues with a high degree of anatomical and cellular accuracy. This level of detail is critical for pharmaceutical development, as it allows scientists to observe how drugs interact with different tissues without relying on animal testing. By mimicking human tissues more closely than ever before, DIP could play a pivotal role in creating safer, more effective therapies.
In the coming years, DIP is poised to bridge the gap between lab research and clinical applications. With its speed, precision, and adaptability, DIP could redefine bioprinting, making it a viable option for a wider range of scientific and medical uses. The team at the University of Melbourne continues to refine the technology, aiming to make it accessible to research facilities worldwide.
This pioneering technology represents a significant step forward in bioprinting, enabling researchers to create viable tissue structures with unprecedented accuracy and efficiency. As it gains traction in the medical field, DIP may become an invaluable tool for advancing medical science and enhancing patient care.
Note: Materials provided above by The Brighter Side of News. Content may be edited for style and length.
Like these kind of feel good stories? Get The Brighter Side of News' newsletter.
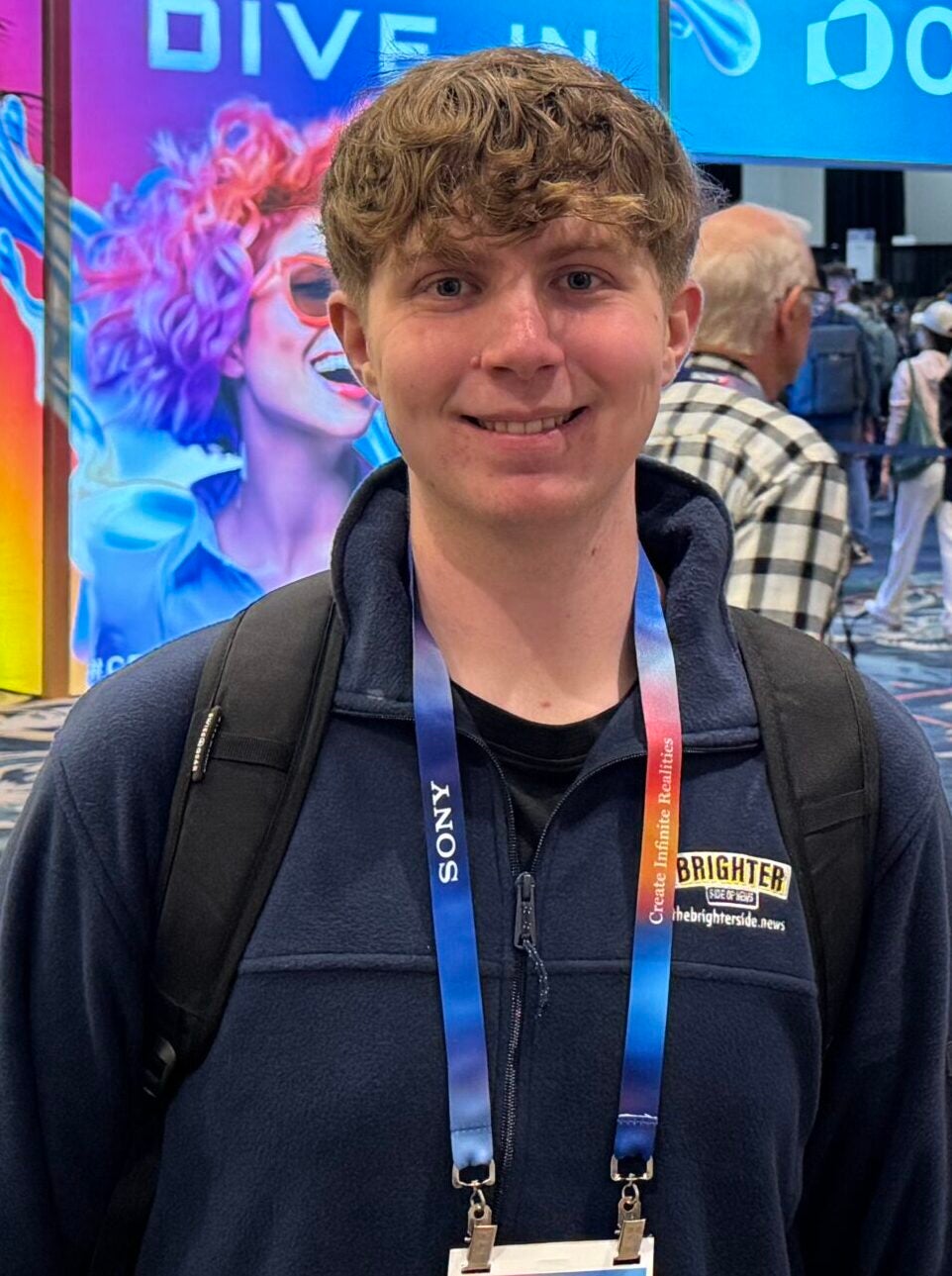
Joshua Shavit
Science & Technology Writer | AI and Robotics Reporter
Joshua Shavit is a Los Angeles-based science and technology writer with a passion for exploring the breakthroughs shaping the future. As a contributor to The Brighter Side of News, he focuses on positive and transformative advancements in AI, technology, physics, engineering, robotics and space science. Joshua is currently working towards a Bachelor of Science in Business Administration at the University of California, Berkeley. He combines his academic background with a talent for storytelling, making complex scientific discoveries engaging and accessible. His work highlights the innovators behind the ideas, bringing readers closer to the people driving progress.